INTRODUCTION
In the 1950s, the Federation of Universities for Animal Welfare (UFAW) initiated a project dedicated to ensuring ethical standards and animal welfare in scientific research, leading to the publication of ‘The Principles of Humane Experimental Technique’1. This marked the establishment of the 3Rs principle, aiming to replace, reduce, and refine the use of mammals in scientific research whenever feasible2,3. Subsequently, laws, guidelines, institutions, and ethics councils were established4.
Currently, numerous alternative methodologies have been proposed as replacements for mammals. These include cell and tissue culture, computer models (such as QSAR – Quantitative Structure-Activity Relationship, and CADD – Computer-Aided Drug Design), lower vertebrates (Danio rerio), lower invertebrates (Drosophila melanogaster, C. elegans, G. mellonella), and microorganisms (Saccharomyces cerevisiae)5,6.
Among the in vivo methodologies for scientific experimentation, G. mellonella stands out for its various advantages: it is cost-effective, requires simple techniques and equipment, and has minimal space requirements for rearing and maintenance. G. mellonella wax provides robust data owing to the resemblance between its immune response, mediated by hemocytes, and the innate immune response of mammals7,8.
G. mellonella, an insect of the order Lepidoptera and family Pyralidae, commonly resides in beehives, primarily feeding on beeswax and pollen during the larval stages9,10. This alternative animal model facilitates swift large-scale studies. Several tests can be conducted using the insect larvae, including survival monitoring, calculation of the median lethal dose (LD50), assessment of pathogen virulence, histopathological examinations, behavioral changes, egg deposition alterations, and immune system adjustments due to infection or chemical substance treatment11.
Studies in this model primarily focus on the larval stage due to the ease of hemolymph extraction for immune system analysis and the manifestation of larval body melanization as an immune response to pathogens and inflammation. Additionally, adult larvae are more manageable for treatment compared to other life stages. Literature reports three forms of larvae treatment: topical, oral, and injectable. Among these, the injectable route has received the most extensive study, followed by the oral route, while the topical route, with fewer investigations, is primarily associated with evaluating fungal virulence12,13.
The immune response observed in G. mellonella primarily involves hemocytes, the most abundant defence cells. Hemocytes facilitate cellular responses via phagocytosis, nodulation, and pathogen encapsulation, while the humoral response involves the production of antimicrobial peptides, phenoloxidase-driven melanization, reactive oxygen species (ROS) production, and hemolymph coagulation8,14.
Phenolic compounds, a subset of exogenous natural antioxidants, constitute secondary metabolites in plants, significantly influencing plant reproduction, morphological development, and physiological processes. Gallic acid (GA), a member of the hydroxybenzoic acid group, stands out as the most important and extensively studied phenolic compound among them15-18. GA possesses diverse beneficial properties, functioning as a potent antioxidant, antimicrobial, antifungal, antiviral, anticarcinogenic, antimutagenic, and anti-inflammatory agent19-22.
Given GA’s therapeutic properties, its multiple applications, and the scarcity of research on the topical route using G. mellonella, this study aimed to evaluate GA’s toxicity in alternative methods, encompassing both in vitro and in vivo assays.
METHODS
This was an experimental study aimed at evaluating the toxicity and permeation of GA using different in vivo and in vitro alternative models, including G. mellonella, C. elegans, and monolayer cell cultures. The study evaluated the toxicity of GA in solution using human keratinocytes (HaCat), human dermal fibroblasts (HDFa), and human liver cell lines (HepG2), in addition to invertebrate animal models for C. elegans and semi-solid formulations in G. mellonella via topical administration.
Compounds
Gallic acid (3,4,5-trihydroxybenzoic acid), 98% purity, was obtained commercially (Dinâmica Química Contemporânea Ltda., Brazil). Stock solutions of GA were prepared in dimethyl sulfoxide (DMSO, Neon) and diluted in Dulbecco Modification of Minimum Essential Media (DMEM, Gibco), Brain Heart Infusion (BHI, Merck), and phosphate-buffered saline (PBS) for experimentation with cell culture, C. elegans, and G. mellonella (injectable administration), respectively.
G. mellonella: in vivo toxicity assays
The assays with G. mellonella larvae were carried out by two pathways treatment, injectable administration (IA) and topical administration (TA). After treatment, these alternative animals were submitted to survival tests, hemocytes quantification tests, and melanization score tests.
For treatment through IA, solutions of GA at 0.5, 1.0, 1.5, 2.0 and 5% were prepared, corresponding to 261.0, 463.0, 695.0 and 920.0 mg/kg of GA injected. For treatment through TA, an emulsion oil/water was prepared (Supplementary file Table 1) and the same concentrations of GA were applied on G. mellonella’s cuticle (261.0, 463.0, 695.0, and 920.0 mg/kg). The emulsion system was prepared by heating the components of oil and water phases separated at 75°C until the complete melting of fatty materials. The aqueous phase was added to the oil phase under manual agitation until it reached ambient temperature, after cooling, GA was added and incorporated by mechanical agitation for 5 min.
The G. mellonella larvae were kept in plastic pots at 25°C, fed with beeswax and pollen until they reached 2–3 cm and mass 180–300 mg. Larvae that had a light color and lack of melanization were selected. Larvae were incubated at 37°C and kept without food in Petri plates for 24 h before the assay. After incubation, larvae were treated, and a Hamilton syringe was used to inject 10 µL of GA solution through IA or with 0.01 g of GA emulsion (which corresponds to the same amount of GA applied in 10 µL of GA solution) through TA following OECD 404 guide for evaluation of acute skin irritation/corrosion of substance in rabbits adapted to G. mellonella larvae23. Groups treated with solution or emulsion without GA were used as negative controls. Topical application of GA emulsion was performed with aid of a spatula in which the emulsion was applied and left for 3 min on the larvae back, then it was removed with a gauze. A second application was performed and maintained for a period of 1 h, then removed. Finally, a third application was made with a duration of 4 h and removed after this time. After treatment, larvae were subjected to the same tests of survival, hemocytes quantification, and melanization.
Survival assay
Groups of 8 to 10 larvae were treated with GA, incubated at 37°C, and death was observed daily for 96 h. Larvae were considered dead when there was no movement and had a dark color11. Lethal dose medium (LD50) was calculated with Graph Prism 5.0 and expressed in mg/kg. Three independent assays were performed with 27 larvae per group.
Hemocytes quantification assay
Groups of 3 larvae were treated with GA and incubated at 37°C for 4 h. After this period, larvae had their hemolymph collected by an incision made on the left side of the last proleg with a scalpel. A pool of each larva was made by removing 2 aliquots of each with 10 µL of hemolymph, then each sample was diluted in anticoagulant solution (2% NaCl; 0.1 M glucose; 30 mM sodium citrate; 10 mM EDTA) in a 1:10 ratio. Finally, two 10 µL aliquots of each sample were vortexed for approximately 1 min and introduced into a Neubauer chamber for quantification of hemocytes using Olympus CH30 microscope24. Three independent assays were performed with 9 larvae per group. The results were expressed as the number of hemocytes/mL.
Melanization assay
Groups of 3 to 4 larvae were treated with GA and after 24 h, the melanization was evaluated following Champion et al.25 by a semi-quantitative method with scores: 0 (complete melanization), 1 (spots black in the brown larva), 2 (less than three spots in line format in the beige larva), and 4 (no melanization). Three independent tests were performed with 9 larvae per group and the results were expressed in a median score of melanization25.
Cell culture: in vitro toxicity assay
Human keratinocytes (HaCat), human dermal fibroblasts (HDFa), and human liver cell lines (HepG2) cells lines were cultured in 75 cm2 culture bottles at 37°C and 5% of CO2 for 3–4 days to adhesion and formation of a monolayer of cells26. After adhesion and confluence of at least 75%, cells lines were treated with trypsin 0.25%, centrifuged at 1500 rpm for 10 min and 5×104 cells per well were pipetted into a 96-well plate. The plates were incubated for 24 h for cell adhesion. Then, they were treated with 100 µL of GA solution in DMEM at concentrations of 7.81–1000 µg/mL. After treatment, cells were incubated for 24 h and 48 h, and 100 µL of a 50 µM resazurin solution in DMEM was added to wells and incubated for 4 h. Finally, cell viability was evaluated by measuring absorbance at 560 nm and 590 nm in a microplate reader (BioTek). The results were expressed as a percentage of living cells26. Inhibitory concentration median (IC50) of GA in each cell line was calculated using Graph Prism 5.0 software. Four independent assays were performed and the results were expressed as mean and standard deviation.
C. elegans: in vivo toxicity assay
The C. elegans AU37 (glp-4; sek-1) strain was cultivated in Petri plates in Nematode Growth Medium (NGM) agar feed with Escherichia coli OP50 and incubated at 15°C. For in vivo toxicity assay, worms were synchronized with 3% sodium hypochlorite until they reached the L4 phase and procedure as in Singulani et al.27. A number of 10–20 worms per well was added in a 96-well plate containing 40% BHI, 60% NaCl, 10 µg/mL of cholesterol in ethanol and antibiotics Kanamycin (Sigma-Aldrich) (90 µg/mL) and Ampicillin (Sigma-Aldrich) (200 µg/mL).
Worms were treated with different concentrations of GA (3.905; 15.62; 62.5; 250; 500; and 1000 µg/mL). Two negative controls (no treatment and DMSO 1%) were used. The plates were incubated at 25°C. Worms were observed daily for 96 h following shape and mobility criteria: sinusoidal worms with movement were considered alive and rod-shaped worms without movement were considered dead. The analysis was performed with ZEISS Axiocam automated microscope ICc 5 and software ZEN 2.3 lite. Three independent assays were performed, with 15 worms per group, and median lethal concentration (LC50) was calculated with Graph Prism 5.0 software.
Statistical analysis
Graph Prism 5.0 (GraphPad Software Inc., CA, USA) was used for statistical analysis. Survival differences for C. elegans and G. mellonella were analyzed by log-rank (Mantel-Cox) test comparing samples to the control group. Hemocytes quantification was performed by ANOVA test with Dunnett’s post-test.
RESULTS
G. mellonella: in vivo toxicity of GA
G. mellonella was used comparing two routes of administration of GA, injectable (GA in PBS solution) and topical (GA incorporated in an O/W emulsion). Figure 1 shows results of survival, hemocytes quantification, and melanization assays.
Figure 1
Galleria mellonella data from gallic acid treatment through injectable administration and topical administration. Larvae survival: A) injectable administration and B) topical administrations. Hemocytes quantification: C) injectable administrations and D) topical administration. Melanization: E) injectable administration and F) topical administrations
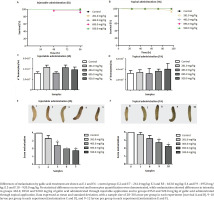
Treatment of G. mellonella wax with GA through IA (Figure 1A) and TA (Figure 1B) did not demonstrate survival differences between control and treated groups, consequently, GA was non-toxic to larvae when present in concentrations from 261.0 to 920.0 mg/kg.
Hemocytes quantification assay was used to verify modifications in cellular immune response in consequence of GA treatment. Both IA (Figure 1C) and TA (Figure 1D) of GA did not demonstrate changes in the number of hemocytes compared to the control group. The number of hemocytes ranged from 3.0×107 to 4.3×107 hemocytes/mL when GA was administrated through IA and varied from 3.18×107 to 4.0×107 hemocytes/mL for TA. The results suggest GA did not change cellular immune response as a consequence of treatment.
Finally, melanization corresponds to the activation of humoral immune response in G. mellonella larvae. Figure 1E shows larvae treated with GA through IA and demonstrates the increased intensity of melanization dose-dependent to GA. Groups treated with GA concentrations of 261.0, 463.0, 695.0 and 920.0 mg/kg had a melanization percentage of 33.3%, 66.6%, 100% and 100% of melanized larvae, respectively, while control group had 0% of melanized larvae. Statistical analysis of melanization data showed significant differences in groups treated with GA concentrations of 463.0, 695.0, and 920.0 mg/kg from the control group (p<0.05). Groups treated with GA through TA, represented in Figure 1F, with concentrations of 261.0, 463.0, 695.0 and 920.0 mg/kg had 8.33%, 25.0%, 41.66%, and 83.33% of the melanized larvae, respectively, and the control group had 0% of melanized larvae. Statistical analysis of melanization data showed significant differences in groups treated with GA concentrations of 695.0 and 920.0 mg/kg from the control group (p<0.05).
HaCat, HDFa, and HepG2 cell lines: in vitro toxicity of GA
Initially, the toxicity of GA at different concentrations was evaluated in three cell lines, two of which represent the skin and one, the liver. GA had no toxicity on all cell lines in concentrations between 7.81 and 62.5 μg/mL (Figure 2). On the other hand, GA at concentrations ≥125 μg/mL led to a reduction in the viability of the three cells in a concentration-dependent way. In addition, the toxic effect of the GA was more pronounced at 48 h compared to 24 h of treatment on the cells.
Figure 2
Cytotoxicity of gallic acid in: A) HaCat, B) HDFa, and C) HepG2 cell lines at 24 and 48 h of analysis
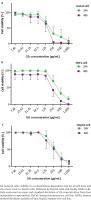
From the graph analysis, we calculated the IC50 and the results showed GA was more toxic to HaCat cell line with IC50 of 182.5 μg/mL and 138.8 μg/mL at 24 and 48 h, respectively (Figure 2A). For the HDFa cell line, GA presented less toxicity with IC50 of 303.4 μg/mL and 247.0 μg/mL at 24 h and 48 h, respectively (Figure 2B). For the HepG2 cell line, GA demonstrated median toxicity with IC50 of 265.1 μg/mL and 207.8 μg/mL at 24 h and 48 h, respectively (Figure 2C).
C. elegans: in vivo toxicity of GA
In addition to in vitro toxicity, C. elegans worms were exposed to GA in BHI solution. The treatment with the lowest concentration (3.905 μg/mL) of GA showed no toxicity in worms (Figure 3). Concentrations of 15.62 μg/mL demonstrated a 59.7% worm survival at 96 h of analysis time. The highest GA concentrations (≥ 62.5 μg/mL) led to the death of most worms in 96 h, ranging from 5.78% to 8.2% of survival at the end of the analysis. Survival curves C. elegans worms exposed to GA concentrations ≥15.62 μg/mL demonstrated statistical differences from the control group (p<0.0001).
By calculating GA’s LC50 for this nematode, we found the value of 16.08 μg/mL at 96 h of treatment. Both negative controls did not present toxicity, having at the end of the assay worm survival ranging from 92.32% to 95.84%.
DISCUSSION
In our study, G. mellonella proved to be a potentially promising alternative model for assessing the toxicity and permeation of natural compounds, particularly through topical administration. This model demonstrated comparable results to other validated methods, offering a viable alternative to traditional mammalian testing.
Alternative methodologies play a pivotal role in replacing the extensive use of animals in conventional toxicity tests. Interspecies variations, such as anatomical, physiological, and biochemical differences, pose a barrier to the direct application of results to humans. Nevertheless, ethical concerns render the use of alternative methods more acceptable in preliminary chemical toxicity tests. Hence, to obtain robust data predicting substance toxicity to humans through alternative methods, integration of results from various in vitro and in vivo tests is imperative28,29. Given GA’s therapeutic properties, this study aimed to evaluate its toxicity using diverse in vitro and in vivo assays.
The toxicity of GA was assessed by the U.S. National Library of Medicine across three different animal species via LD50, utilizing rats, mice, and rabbits as models through various administration routes. For instance, the LD50 for rats via the subcutaneous route was 5 mg/kg30. Similarly, oral administration of GA in rabbits resulted in an LD50 of 5 mg/kg, mirroring the result observed in rats upon subcutaneous GA administration31. A significant amount of GA was necessary for the observed harmful effects through subcutaneous, intraperitoneal, and oral routes in these models. Notably, the intravenous route demonstrated a significantly lower lethal dose compared to other administration methods in mice.
In a recent study by Variya et al.32, the subacute toxicity of repeated oral doses of GA for 28 days in albino mice concluded that the lethal dose for 50% of animals due to GA exceeded 2000 mg/kg, with an intake of 900 mg/kg/day showing no hematological, morphological, or histopathological alterations in this model. Similarly, van der Heijden et al.33 reported that GA metabolizes in rats through the liver and is primarily excreted through urine, without any observed toxicity even at high doses. This suggests GA’s potential use as an antioxidant in foods or in combination with other antioxidants. Additionally, oral administration of GA did not produce toxic effects in pigs and dogs at doses ranging from 117 to 5000 mg/kg33.
The present study introduced G. mellonella larvae as another in vivo model. Allegra et al.34 proposed G. mellonella as a methodology to identify toxic and non-toxic substances due to variations in hemocyte responses. Our comparative analyses in this study included survival, hemocyte count, and melanization in G. mellonella through injectable and topical routes.
Injectable administration (IA) of GA in G. mellonella larvae showed no significant discrepancies in the survival test between treated and control groups at concentrations ranging from 50 to 200 µg/larva of GA. Similarly, no notable changes in the hemocyte count were observed as a result of GA treatment at the proposed concentrations. However, melanization quantification showed statistical discrepancies at higher GA concentrations, indicating the activation of the humoral response in these larvae.
In the topical administration (TA), GA was incorporated into an oil/water emulsion due to its low solubility in water and its hydroxyl groups (-OH) attached to the aromatic ring. While no data were available in the literature demonstrating GA’s toxicity in G. mellonella larvae, our study innovatively administered GA topically. The survival test revealed no statistical differences between GA treatment and control groups, signifying non-toxicity at the tested concentrations. Similarly, the hemocyte quantification showed no significant differences between treated and control groups, whereas melanization quantification revealed increased melanization with higher GA concentrations. Comparison between IA and TA highlighted increased melanization in larvae from injectable treatments, suggesting a higher humoral response activation compared to topical administration.
Instead of using mammalian animals for toxicity tests, cellular models have become a crucial means to predict harm to humans. These models have made significant advancements alongside technology, resulting in robust methodologies35.
For instance, GA’s cytotoxicity in HaCat cells displayed increased toxicity with prolonged treatment time. At 24 h post-treatment, the IC50 was 182.5 µg/mL, which decreased to 138.8 µg/mL at 48 h. The concentration-dependent nature of GA’s toxicity to HaCat cells was evident. These findings align with the study by Yang et al.36, which evaluated GA effects on human keratinocytes, concluding that concentrations above 200 µg/mL altered cell viability. However, lower concentrations (10, 20, or 50 µg/mL) did not affect living cell percentages after 24 h of treatment36.
The current research trend is the evaluation of toxic properties in plant extracts containing GA. For example, Varma et al.37 assessed the toxicity of Triphala extract, a blend of three different plants rich in Ayurvedic medicine, predominantly composed of GA, ellagic acid, and chebulinic acid. This compound, comprising approximately 34.6 ± 3% phenolic acids (including gallic acid), demonstrated an IC50 of 239.16 ± 4.3 µg/mL in HaCat cells, closely aligning with the results from previous studies36,37.
Additionally, the present study revealed that HDFa cells treated with 250 µg/mL of GA exhibited 69.1% cell viability after 24 h. Subsequent calculation of the inhibitory concentration of 50% of the cells showed values of 303.4 µg/mL and 247 µg/mL at 24 h and 48 h, respectively. These results were similar to a previous study where concentrations of 200 µg/mL of GA resulted in approximately 70% cell viability in HDF cells, indicating lower cell viability at higher concentrations38.
In another study by Silva et al.39, the cytotoxicity of semisynthetic esters derived from GA in the HepG2 cell line suggested that the IC50 of respective esters occurred at concentrations of 200, 145, 42, and 30 µg/mL. Furthermore, Tanaka et al.40 found GA’s ability to inhibit lipid accumulation in HepG2 cells through the adenosine monophosphate-activated protein kinase (AMPK) pathway without exhibiting cytotoxicity at the tested concentrations.
At concentrations of 265.1 µg/mL, 50% of the cells died in 24 h treatment, highlighting that beneficial effects of GA were overpowered by the observed toxicity at concentrations exceeding 200 µg/mL. Interestingly, the results in the HepG2 cells indicated that GA’s toxicity was notably lower than its gallates, where a hydroxyl group, not a carbon chain, functioned as the substituent39.
Comparing the IC50 results across the three cell lines studied in this research revealed that HDFa cells exhibited lower sensitivity to GA than HepG2 cells, with HaCat cells manifesting the highest sensitivity to the compound.
C. elegans was included in this study due to the larvae’s cuticle similarity to human skin, allowing comparison with cell lines and G. mellonella’s cuticle41. Although literature evaluating GA’s toxicity in this model is scarce, the present study used it for its correlation with mammalian toxicity and lower testing costs42.
Hunt43 differentiates C. elegans from cellular models, citing its complete model status, predicting toxicity in mammals through neural, motor, digestive, and sensory responses. Boyd et al.42 noted that incorporating C. elegans in toxicity studies enhances reliability and safety in predicting toxic effects in humans. Hence, the inclusion of C. elegans in GA toxicity assessment is crucial for correlating data across various mammalian methodologies42.
Singulani et al.27 analyzed the antifungal activity of GA and its gallates in C. elegans infected with Candida albicans, suggesting that gallates’ low antifungal efficacy at high concentrations could be linked to their toxicity in this alternative animal model. Pedroso et al.44 investigated the antifungal activity of plant extracts in C. elegans, discovering that GA at high concentrations caused larval deaths within four days, supporting the idea that elevated GA concentrations lead to toxicity in this model.
Considering the alternative mammalian method, Danio rerio (zebrafish) at the embryonic stage, Boyd et al.42 established a correlation between LC50 results in C. elegans and D. rerio. The zebrafish, known for its relevance in genetic, developmental, toxicological, and pharmacological research, showcased no toxicity from GA at concentrations between 1 and 120 µg/mL27. Additionally, Harishkumar et al.45 reported an LC50 of 304 µg/mL for GA in fish.
Comparing the LC50 results between C. elegans and D. rerio indicated approximately 19 times more toxicity in C. elegans compared to the fish. Although physiological and biochemical correlations explaining this discrepancy are lacking, both alternative methods are instrumental in assessing chemical toxicity and predicting mammalian toxicity42.
The use of G. mellonella as an alternative methodology is significant as it allows for comparisons with mammalian methods. The present study’s results emphasize the tolerance of G. mellonella to GA compared to mammalian methodologies, suggesting that higher doses were required for observed toxic effects in these animals, whereas we tested doses up to 920 mg/kg. Exploring new in vitro and in vivo methods for potential compounds such as GA is crucial for assessing toxicity in mammals.
Limitations
Notably as a study limitation, while alternative models, such as G. mellonella and C. elegans, contribute significantly to the 3Rs principle (replace, reduce, refine) in scientific research, they cannot fully replace mammalian models in advanced stages of testing, such as phase III and IV studies. However, these models may play a crucial role in minimizing the use of mammals in earlier phases of toxicity and efficacy testing.
CONCLUSIONS
This research aimed to validate G. mellonella as an alternative model for toxicity testing, comparing its results with other validated methodologies. Toxicity of gallic acid (GA) was assessed through survival evaluation, hemocyte quantification, and melanization in G. mellonella, but the LD50 could not be determined when administered by injection or topical application. The oil/water emulsion proved to be more suitable for topical administration than the aqueous gel formulation. Injectable GA did not affect survival or cellular immune response, though humoral responses were affected at concentrations >463 mg/kg. Topical administration in an oil/water emulsion had a similar effect, with changes in humoral response occurring at concentrations >695 mg/kg. Compared to other models, C. elegans was more sensitive to GA, followed by HaCat, HepG2, and HDFa cells, while G. mellonella showed no toxicity at the tested concentrations. GA’s cytotoxicity increased over time in cell cultures, requiring lower concentrations to produce similar effects at 48 h compared to 24 h. C. elegans required approximately seven times lower concentrations than cell models to achieve 50% mortality. Overall, the alternative methods using cell cultures and C. elegans were viable for GA toxicity assessment. G. mellonella showed promise for future studies in topical testing, though further research is needed to fully validate its use in toxicity assays.